The global climate is undergoing major changes due to high concentrations of carbon dioxide in the atmosphere [1]; this is already evident from the occurrence of more extreme weather events around the world [2]. Energy consumption also induces climate change, but at the same time, climate change impacts the energy sector, both in terms of supply capacity and changes in energy demand [3].
The building sector, one of the most energy-intensive, will be strongly affected by this change. Buildings are recognized as the largest contributors to global warming [4] and are responsible for a large proportion of total energy consumption [5], followed by transportation, industry, and agriculture. Governments have begun revising their energy strategies and policies to mitigate these problems, and are working to achieve zero net greenhouse gas emissions by 2050. One answer to mitigating or rather reducing this trend is the different types of renewable energies that are increasingly widespread [6],[7],[8]. There is a growing interest in energy-efficient environmental systems that guarantee an adequate indoor climate for years to come. By increasing building energy efficiency, significant economic, social, and environmental benefits can be achieved [9].
Reducing building energy demand requires a broad awareness of how the climate will change to direct effective building energy efficiency actions [10]. This applies both to the design of new buildings and in the retrofit phase. It is unthinkable that actions taken today will be ineffective, due to climate change, in the next 50 years, when the investment costs have not yet been amortized.
A large part of primary energy is used for heating and cooling buildings [11], [12]. It is expected that in the future cooling energy demand will prevail over heating [13]. An increase in outdoor temperatures can lead to a significant worsening of indoor comfort in buildings. This results in the need for air conditioning systems, leading to increased energy consumption and operating costs. This is especially true for buildings constructed in today's warm climates where a correct choice of air conditioning systems can contribute to climate change mitigation [14], although it is a very complex assessment, as many variables must be considered [15]. The key questions to ask, as well as the starting point for this work, are: will these technologies be effective even with the coming climate changes? How will their performance vary in different climate zones around the world? Will the investment require to install them be recovered in a reasonably short time?
In this context, air-source heat pumps (ASHPs) have captured growing interest due to their high energy efficiency. The ASHP is a technology that can significantly contribute to the reduction of energy consumption in buildings. Among all types of heat pump systems, the ASHP has many advantages [16], it is an efficient and environmentally-friendly system that can provide an adequate indoor climate [17], and it is a viable alternative to traditional air conditioning systems, both in terms of energy and cost. Although these systems are widely adopted, their performances are strongly affected by external conditions, especially in cold areas [18]. Specifically, the performance of ASHP systems is significantly reduced below low temperatures [19].
In a previous study, air pre-treatment before meeting the ASHP, using a geothermal system, was shown to improve the winter and summer performance of the heat pump [20]. Pre-treatment leads to a more noticeable improvement in extremely cold climates. The pre-treatment reduces the heat pump shutdown periods when the outdoor temperature is below the operating limit temperature. The result of combining the air source heat pump with the geothermal air probe is to extend the use of the heat pump where it is currently not cost-effective, as the average outdoor temperature is very often below the operating limit temperature of the heat pump [21].
Shen et al. [22] already showed the negative influence of climate change on the efficiency of a ground source heat pump due to the increase in water temperature at the inlet and outlet of the heat pump.
Several forecasting tools for generating future climate data have been developed. They allow the generation of future climate data on an hourly and annual basis for many cities around the world. There are several approaches in the literature for climate change analysis, and Moazami et al. [23] provided a comparison of the major databases. Among the most widely used ones, the most popular are CCWorldWeatherGen, WeatherShift™, and Meteonorm. The CCWorldWeatherGen [24] computational software provides climate data over a wide time interval through the morphing procedure and input data supplied by EnergyPlus [25], [26]. The morphing sequence matches recorded climate data with climate models, generating time series containing weather conditions from future climate scenarios, including the IPCC's A2 greenhouse gas emissions framework. The CCWorldWeatherGen creates future weather files for three-timeframes: 2020 (which runs from 2011 to 2040), 2050 (from 2041 to 2070), and 2080 (from 2071 to 2100). The procedure aims to "scale" the climate model data in proportion to the recorded data, providing the required spatial and temporal data with high accuracy.
The objective of this work is to analyze the behaviour of a heat pump considering different climate scenarios. For this purpose, two locations have been selected, Yakutsk (Sakha Republic, Russia) and Damascus (Syria), characterized by extremely different climates. Once the seasonal behaviour of the heat pump was analyzed considering the current climate conditions, a possible future scenario due to climate change was analyzed. Climatic data for 2050 and 2080 have been extracted from the CCWorldWeatherGen software, in order to evaluate the impact of climate change on the heat pump behaviour in both climate zones. Heat pump performance was investigated by calculating the Seasonal Coefficient of Performance (SCOP), and Seasonal Energy Efficiency Ratio (SEER).
SCOP is the seasonal coefficient of performance, which indicates the operating conditions of the ASHP, through the ratio of the annual heating demand to the annual electricity consumption dedicated to heating. Similarly, SEER is the seasonal energy efficiency coefficient, related to cooling. The SCOP and SEER are carried out following UNI EN 14825. These seasonal indices have been calculated on an hourly basis, varying the geographical area and, therefore, the outdoor temperature and the water supply temperature to the user.
The SCOPon is the active seasonal coefficient of performance, which is the coefficient of performance of the unit in active mode for the designated heating season. It is determined by the part load, the supplemental heating capacity (where required), and the interval coefficients of performance, weighted by the hourly intervals at which the interval regime occurs.
SCOPnet is the net seasonal coefficient of performance, which is the seasonal performance of a unit in the active heating mode without supplemental heaters.
The SEERon is the seasonal energy efficiency ratio in active mode. It indicates the average energy efficiency of the unit in active mode for the cooling function and is determined by the part load and the efficiency coefficients for the specific ranges, weighted by the hourly ranges in which the interval regime occurs.
This paper proposes a detailed analysis of the performance of an ASHP considering long-term climate change in two very different climates. The following paragraphs provide details about the heat pump selected as a case study and the two locations chosen. The SCOP and SEER of the heat pump are calculated considering current and future climate data.
The chosen heat pump is a single-circuit air-cooled unit, suitable to meet the heating and cooling needs of medium and small users in residential or commercial buildings. It is has maximum thermal capacity of 30 kW. The study is based on a single heat pump installed in different locations. The objective is to test its behaviour in different climate scenarios, therefore this choice reduces the variables of the problem and provides an overview of the effect of climate change on hours of operation in very different locations.
The main components of the ASHPs are high-efficiency scroll compressors, consisting of a permanent magnet DC motor (high-side type) for variable speed operation, axial fans, a plate heat exchanger, and an aluminium finned coil. The inverter technology allows the heating and cooling capacity to be modulated continuously from 25% to 100% and to instantly adapt the supplied capacity to the needs, providing an average energy saving of 20% compared to a traditional heat pump on/off.
In winter, ASHP heats water up to 35 °C for low-temperature heating systems such as radiant floors and/or up to 50 °C for medium-temperature heating systems such as fan coils. In summer, ASHP, working as a chiller, cools the water down to 7 °C and 18 °C.
The operation of the heat pump depends on the outside temperature. The equilibrium temperature is 16 °C.
In winter:
for the water heating at a temperature (Tw) of 35 °C, ASHP is on when outside temperatures range from -15 °C to 16 °C;
for Tw of 50 °C, ASHP is switched on when the outside temperature ranges from -1 °C to 16 °C.
In summer:
for Tw of 7 °C, ASHP is on when outside temperatures range from 16 °C to 46 °C;
for Tw of 18 °C, ASHP is switched on when the outside temperature ranges from 16 °C to 40 °C.
The behaviour of the ASHP is investigated in Yakutsk and Damascus, selected for their very different climates. In accordance with the Köppen-Geiger classification, Yakutsk is classified as an extremely cold subarctic climate (Dfd), and Damascus a cold desert climate (Bwk). Climate data for 2020, 2050, and 2080 are extrapolated from CCWorldWeatherGen calculation software.
Figure 1 and Figure 2 show the hourly and yearly trends of the external air temperature of Yakutsk and Damascus over the years, respectively.
Table 1 shows the maximum, minimum, and average outdoor temperature values for the years 2020, 2050, and 2080s. Moving from 2020 to 2080 there is an increase in maximum outdoor temperatures of 3 °C in both Yakutsk and Damascus. It is very evident the temperature increase of 5.4 °C of the minimum outdoor temperatures in Yakutsk, while in Damascus it is 1.8 °C. The average temperature, always passing from 2020 to 2080, increases by 4.3 °C in Yakutsk and 2.7 °C in Damascus.
Hourly and yearly trends of the external air temperature of Yakutsk over the years
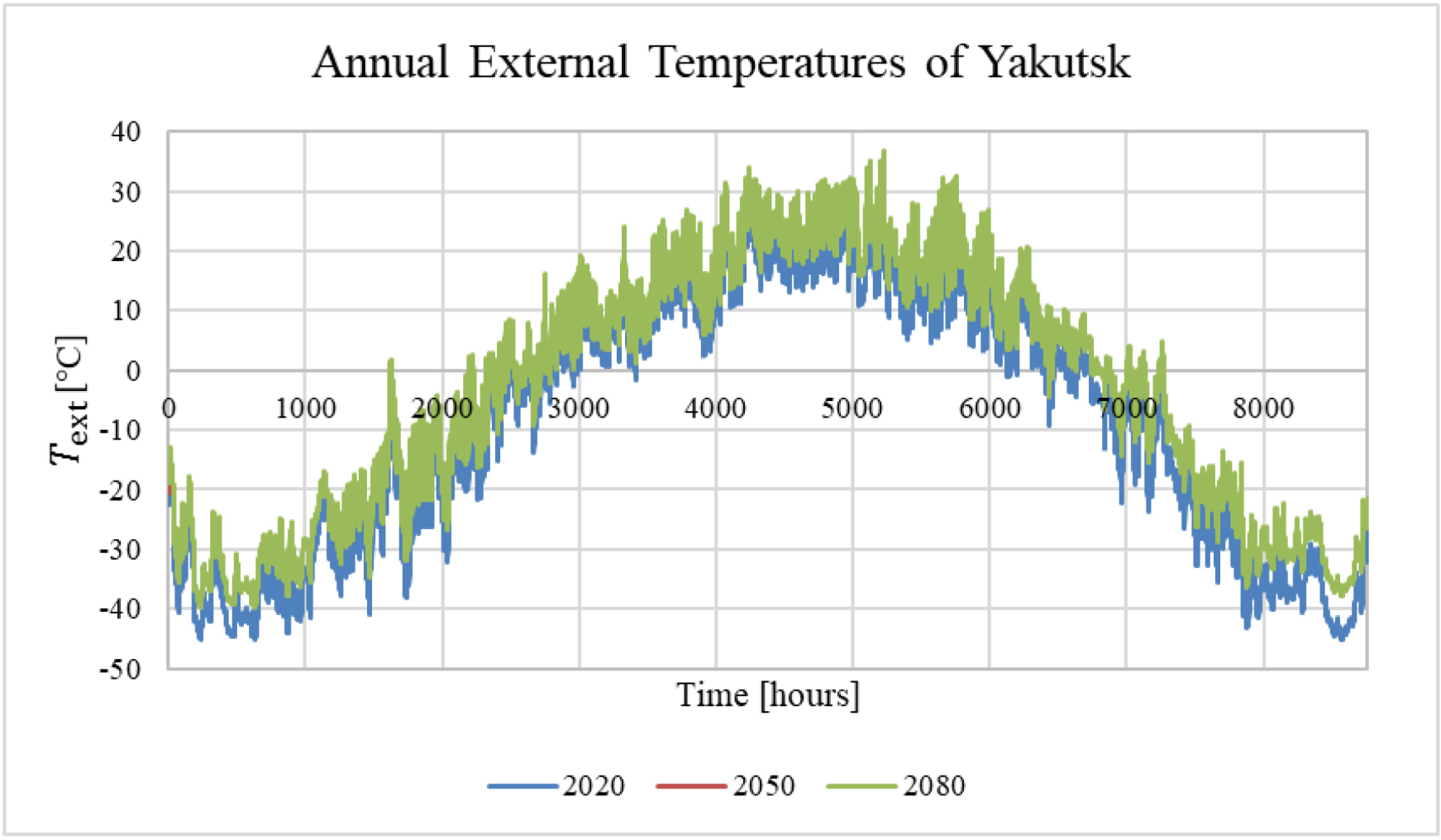
Hourly and yearly trends of the external air temperature of Damascus over the years
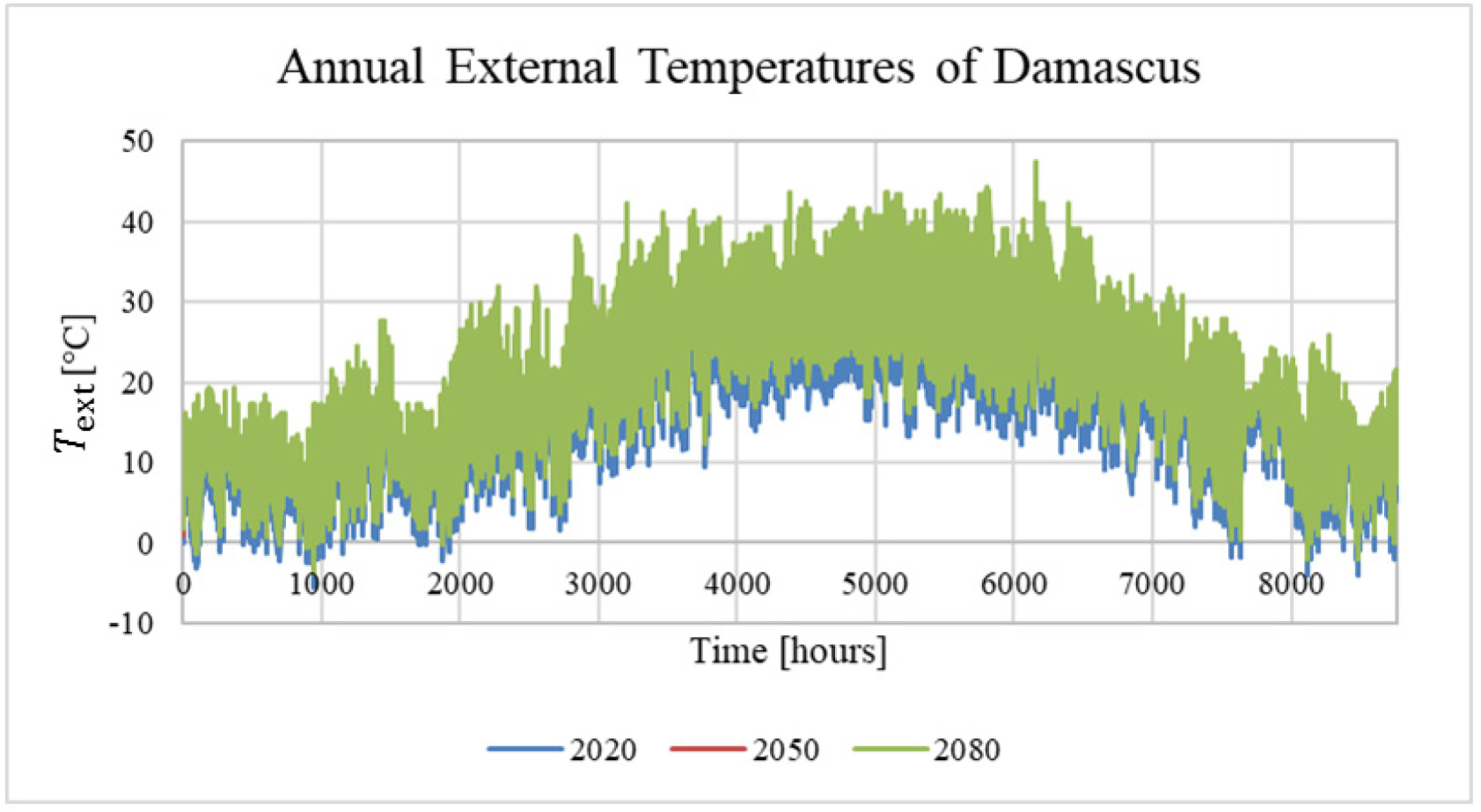
Maximum, minimum and average external temperature of the cities of Yakutsk and Damascus, during the years
Text [°C] |
||||||
---|---|---|---|---|---|---|
Yakutsk |
Damascus |
|||||
2020 |
2050 |
2080 |
2020 |
2020 |
2020 |
|
MAX |
33.9 |
35.5 |
36.9 |
44.5 |
45.6 |
47.5 |
MIN |
-45.2 |
-42.9 |
-39.8 |
-5.4 |
-4.7 |
-3.6 |
AVERAGE |
-7.5 |
-5.5 |
-3.2 |
17.6 |
18.6 |
20.3 |
This section presents the results of the current and future behaviour of the same heat pump at two selected locations. SCOP and SEER are calculated following the procedure proposed by UNI EN 14825.
The winter behaviour of the heat pump is examined through SCOPon (Table 2) and SCOPnet (Table 3) calculations, for Yakutsk and Damascus, considering the years 2020, 2050, and 2080. The heat pump is evaluated for the water heating at a temperature (Tw) of 3 °C and 50 °C.
SCOPon values of the ASHP for Yakutsk and Damascus, over the years
SCOPon |
||||||
---|---|---|---|---|---|---|
Tw = 35 °C |
Tw = 50 °C |
|||||
2020 |
2050 |
2080 |
2020 |
2050 |
2080 |
|
Yakutsk |
2.08 |
2.04 |
2.03 |
1.67 |
1.67 |
1.66 |
Damascus |
2.30 |
2.27 |
2.21 |
1.78 |
1.77 |
1.76 |
SCOPnet values of the ASHP for Yakutsk and Damascus, over the years
SCOPnet |
||||||
---|---|---|---|---|---|---|
Tw = 35 °C |
Tw = 50 °C |
|||||
2020 |
2050 |
2080 |
2020 |
2050 |
2080 |
|
Yakutsk |
2.29 |
2.28 |
2.28 |
1.74 |
1.74 |
1.75 |
Damascus |
2.30 |
2.27 |
2.21 |
1.78 |
1.77 |
1.76 |
The results show that SCOPon and SCOPnet values decrease from 2020 to 2080. The decrease is not very marked for both cities. The highest SCOPon and SCOPnet values are in Damascus, which is the warmest city. Moving from 2020 to 2080, the decrease in SCOPon and SCOPnet is slightly greater for Tw of 35 °C, compared to Tw of 50 °C.
Observing the values of SCOPon and SCOPnet for the two different cities, for the city of Damascus, the two values coincide, whereas for Yakutsk they differ. This is explained by the condition that must be met for the value of electric heating capacity elbu (Tj).
The bin method requires the availability of the hourly frequencies at which outdoor air temperatures occur during the considered season in a given climate. The range of possible outdoor temperatures is divided into temperature intervals, with a width of 1 °C. The frequency curve is plotted, expressing the time the temperature is within the interval (defined as bin). Then the cumulative curve is plotted, expressing the number of hours the temperature is above the bin interval.
The elbu(Tj) represents the auxiliary heating capacity by a heater supplementing the declared capacity for heating when the capacity of the unit Pdh(Tj) is lower than the heat load Ph(Tj) for a specific bin temperature Tj.
If:
(1)
(2)
where Pdh(Tj) is the declared output of the heat pump relative to temperature Tj and Ph(Tj) is the heating part load relative to temperature Tj.
This value of elbu(Tj), in the case that for each temperature value Tj is equal to zero, makes equal the numerators of the relations expressing the calculation of SCOPon and SCOPnet of a heat pump. In fact, in the city of Damascus this condition occurs, the two calculation relations SCOPon and SCOPnet coincide.
Table 4 reports the ASHP winter operating hours. It is interesting to note that as the years go on, the hours of operation of the ASHP decrease. This is happening in both climates due to global warming.
ASHP winter operating hours
Winter - operating hours |
||||||
---|---|---|---|---|---|---|
Tw = 35 °C |
Tw = 50 °C |
|||||
2020 |
2050 |
2080 |
2020 |
2050 |
2080 |
|
Yakutsk |
3663 |
3614 |
3514 |
3476 |
3349 |
3266 |
Damascus |
4079 |
3753 |
3200 |
4079 |
3753 |
3200 |
Looking at Table 4, for the city of Yakutsk, the hours of operation of the heat pump are lower than in the city of Damascus. This happens because the heat pump is suitable for cities with medium or warm climates, while for cold cities it is not advisable. In fact, in the cold city, very often the temperature drops below the limit temperature values, and the system is switched off for many hours.
From 2020 to 2080 in Yakutsk the decrease in operating hours of ASHP in the winter regime for Tw of 35 °C is 149 hours, and for Tw of 50 °C is 210 hours. Also moving from 2020 to 2080 in Damascus, the decrease in winter operating hours for both Tw of 35 °C and Tw of 50 °C is 879 hours.
Table 5 shows the non-operating hours of the system, i.e., when in winter the temperature drops below -15 °C (for Tw of 35 °C) and below -12 °C (for Tw of 50 °C). In winter, there is a significant reduction in the number of non-operating hours of the system in the cold climate of Yakutsk over the years, while in the city of Damascus the system is always operating.
Specifically, in Yakutsk, going from 2020 to 2080 the ASHP non-operating hours for Tw of 35 °C decrease by 413 hours, and for Tw temperature of 50 °C the non-operating hours decrease by 330 hours.
ASHP winter non-operating hours
Winter - non-operating hours |
||||||
---|---|---|---|---|---|---|
Tw = 35 °C |
Tw = 50 °C |
|||||
2020 |
2050 |
2080 |
2020 |
2050 |
2080 |
|
Yakutsk |
3482 |
3248 |
3069 |
3737 |
3598 |
3407 |
Damascus |
0 |
0 |
0 |
0 |
0 |
0 |
Similarly, in Table 6, the analyses are performed for SEERon. For both cities, SEER increased over the years due to global warming. In Damascus, the values are higher. Specifically, from 2020 to 2080 the SEERon increases by 0.09 at Yakutsk (both for Tw equal to 7 °C and 18 °C), at Damascus it increases by 0.02 for Tw equal to 7 °C and 0.02 for Tw equal to 18 °C is 0.05.
SEERon values of the ASHP for Yakutsk and Damascus, over the years
SEERon |
||||||
---|---|---|---|---|---|---|
Tw = 7 °C |
Tw = 18 °C |
|||||
2020 |
2050 |
2080 |
2020 |
2050 |
2080 |
|
Yakutsk |
1.92 |
1.98 |
2.01 |
2.19 |
2.25 |
2.28 |
Damascus |
2.14 |
2.16 |
2.16 |
2.49 |
2.52 |
2.54 |
Table 7 reports the ASHP summer operating hours. It is evident how over the years there will be an increase in ASHP operating hours, even in a cold climate.
ASHP summer operating hours
Summer - operating hours |
||||||
---|---|---|---|---|---|---|
Tw = 7 °C |
Tw = 18 °C |
|||||
2020 |
2050 |
2080 |
2020 |
2050 |
2080 |
|
Yakutsk |
1550 |
1817 |
2110 |
1550 |
1817 |
2110 |
Damascus |
4682 |
5008 |
5560 |
4663 |
4963 |
5392 |
Moving from 2020 to 2080 in Yakutsk, an increase in summer operating hours of 560 hours is observed for both Tw of 7 °C and Tw of 18 °C. The increase in operating hours is greater in Damascus and, for Tw of 7 °C, are equal to 878 hours and, for Tw of 18 °C, are equal to 729 hours.
Figure 3 shows the annual energy consumption for winter and summer air conditioning, with radiant floor systems (Tw = 35 °C) and fan-coils (Tw = 50 °C) for the two cities with scenarios of 2050 and 2080.
For the city of Yakutsk, there is little change in energy consumption for winter air conditioning but a significant increase in consumption for summer air conditioning of more than 65%.
For the city of Damascus, there is a significant reduction in consumption for winter air conditioning of about 30% and an increase in consumption for summer air conditioning of about 30%.
Figure 4 highlights a substantial increase in energy consumption related to climate change in the coming years. The biggest increases are for the city of Yakutsk, in particular, better performance of the fan-coil system in summer air conditioning is noted.
Annual electricity consumption [kWh] for heating and cooling
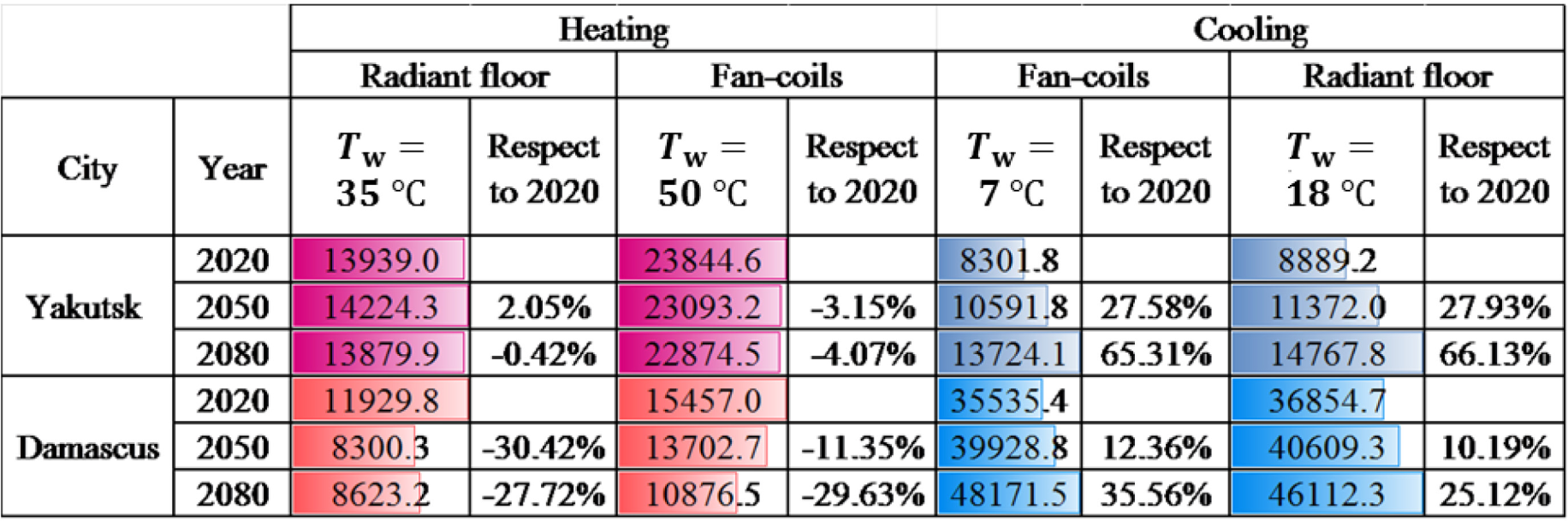
Annual electricity consumption [kWh] for air conditioning
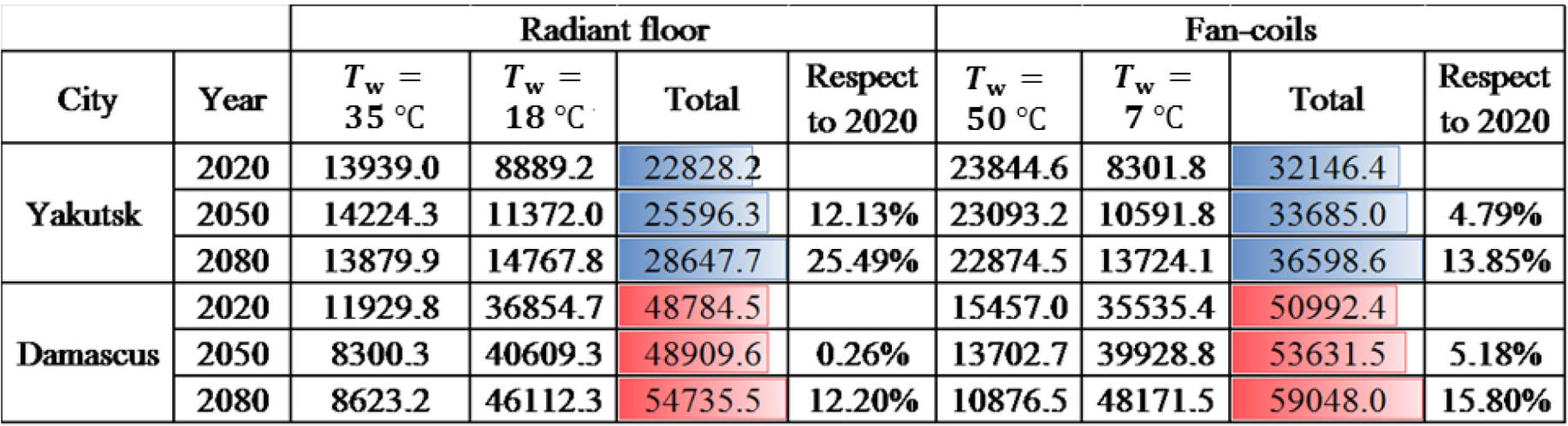
From the analysis of the results, it can be seen that under extreme weather conditions, either too low or too high temperatures, the heat pump goes to safety and turns off. In such cases, it is necessary to provide back-up systems to ensure winter and summer heat demand coverage. In the summer regime, it is plausible to provide for a possible condensing boiler to make up the heat output deficit; in summer regime, several solutions are possible, such as resorting to geothermal heat pumps, which work with more moderate temperatures by virtue of heat exchange with the ground, or absorption heat pumps.
It is expected an increasing global temperature caused by climate change. Climate change emerges as a very important issue, especially in the building sector. Systems increasingly resilient to this change are required. Accelerating energy demand and depletion of fossil fuels have dramatically changed the global energy landscape. Globally, there has been a shift towards the use of renewable energy sources to mitigate environmental crises resulting from climate change.
A great deal of attention is paid to air-source heat pumps, which are an optimal solution from energy, and environmental points of view. Although their use is widespread, it is necessary to take into account that their operation is highly influenced by external weather conditions. One of the major issues in their use is mainly in cold climates, where outdoor temperatures are too low.
This study aims to provide an overview of the future behaviour of air source heat pumps considering climate change. The chosen heat pump has a maximum thermal capacity of 30 kW, its performances are tested in different locations. The choice of testing the same heat pump permits a reduction of variables of the problem and provides an overview of the effect of climate change on hours of operation in very different locations.
The selected cities for the installation of the heat pump are Yakutsk and Damascus, characterized respectively by extremely cold subarctic and cold desert climates. This study analyzes the behaviour of the heat pump in the current and future climate, analyzing the years 2020, 2050, and 2080.
The behaviour of the heat pump has been analyzed by calculating the Seasonal Coefficient of Performance (SCOP), and Seasonal Energy Efficiency Ratio (SEER) according to UNI EN 14825. Moreover, to evaluate the increase or reduction in the use of the heat pump in different scenarios, the actual hours of operation of the heat pump during the year have been calculated.
The results show that SCOPon and SCOPnet values decrease from 2020 to 2080. The decrease is not very marked for both cities, it is slightly greater for Tw of 35 °C, compared to Tw of 50 °C. The highest SCOP values are in Damascus, which is the warmest city. For the city of Yakutsk, the hours of operation in winter of the heat pump are lower than in the city of Damascus. This happens because the heat pump is suitable for cities with medium or warm climates, while for cold cities it is not advisable. In fact, in a cold city, very often the temperature drops below the limit temperature values, and the system is switched off for many hours.
For both cities, SEER increased over the years due to global warming. In the city of Damascus, the values are higher. In the summer, there will be an increase in ASHP operating hours over the years, even in a cold climate.
In conclusion, this study shows that over the years, for both cities, there is an overheating that leads to less wide frequency distributions for winter temperatures and wider frequency distributions for summer temperatures. This leads, over the years, to a slight attenuation of SCOP values and a reduction in the hours of operation of the heat pump for heating. While for cooling there is a slight increase in SEER values and an increase in the hours of operation in different years.
Due to climate change, the performance of heat pumps will vary differently in different geographical areas. Winter operating hours are expected to increase in very cold climates and decrease in temperate climates. This will force air source heat pumps to work under extreme conditions for longer periods in hot climates. Thus it will tend to be observed that there will be wide use of these systems in cold climates, where they could not be used in the past because winters were too harsh, and a reduction in areas that are too warm and will become even warmer in the future.
According to this study, climate change may facilitate the switch from fossil fuel generators to electric heat pumps during winter. Very hot climatic areas may experience automatic shutdowns of heat pumps due to too high outdoor temperatures during the summer season. It is therefore necessary to implement passive energy efficiency measures in the building envelope. For instance, plant systems can be minimized through improved solar gains control in the envelope.
This study could be extended to all climates around the world to return a comprehensive predictive mapping of heat pump performance as a consequence of climate change.
elbu(Tj) |
required capacity of an electric supplementary heater for the corresponding temperature Tj |
[kW] |
Pdh |
declared capacity in heating mode |
[kW] |
Ph(Tj) |
part load for heating |
|
SCOPnet |
net seasonal coefficient of performance |
[-] |
SCOPon |
active mode seasonal coefficient of performance |
[-] |
Text |
external temperature |
[°C] |
Tj |
bin temperature |
[°C] |
Tw |
temperature of water production |
[°C] |
Abbreviations |
||
ASHP |
Air-Cooled Heat Pump |
|
SEER |
Seasonal Energy Efficiency Ratio |
Long-term predictive energy analysis of a high-performance building in a mediterranean climate under climate change ,Energy , Vol. 238 ,pp 121641 , , https://doi.org/https://doi.org/10.1016/j.energy.2021.121641
, Impact of Climate Change on the Backup Infrastructure of Highly Renewable Electricity Systems ,Journal of Sustainable Development of Energy, Water and Environment Systems , Vol. 6 (4),pp 710–724 , , https://doi.org/https://doi.org/10.13044/j.sdewes.d6.0209
, Climate Change and Vulnerabilities of the European Energy Balance ,Journal of Sustainable Development of Energy, Water and Environment Systems , Vol. 3 (1),pp 106–117 , , https://doi.org/https://doi.org/10.13044/j.sdewes.2015.03.0008
, Worldwide dynamic predictive analysis of building performance under long-term climate change conditions ,Journal of Building Engineering , Vol. 42 ,pp 103057 , , https://doi.org/https://doi.org/10.1016/j.jobe.2021.103057
, Achieving zero-energy building performance with thermal and visual comfort enhancement through optimization of fenestration, envelope, shading device, and energy supply system ,Sustainable Energy Technologies and Assessments , Vol. 44 ,pp 101020 , , https://doi.org/https://doi.org/10.1016/j.seta.2021.101020
, A literature review and statistical analysis of photovoltaic-wind hybrid renewable system research by considering the most relevant 550 articles: An upgradable matrix literature database ,J Clean Prod , Vol. 295 ,pp 126070 , , https://doi.org/https://doi.org/10.1016/j.jclepro.2021.126070
, Renewable energy and climate change ,Renewable and Sustainable Energy Reviews , Vol. 158 ,pp 112111 , , https://doi.org/https://doi.org/10.1016/j.rser.2022.112111
, - , Hourly forecasting of the photovoltaic electricity at any latitude using a network of artificial neural networks
Design Optimization of Energy Efficient Residential Buildings in Mediterranean Region ,Journal of Sustainable Development of Energy, Water and Environment Systems , Vol. 10 (2),pp 1–21 , , https://doi.org/https://doi.org/10.13044/j.sdewes.d9.0385
, Dynamic Evaluation of the Effects of Climate Change on the Energy Renovation of a School in a Mediterranean Climate ,Sustainability , Vol. 13 (11),pp 6375 , , https://doi.org/https://doi.org/10.3390/su13116375
, Experimental validation of horizontal air-ground heat exchangers (HAGHE) for ventilation systems ,Geothermics , Vol. 80 , 2019, https://doi.org/https://doi.org/10.1016/j.geothermics.2019.02.010
, Horizontal Air-Ground Heat Exchanger Performance and Humidity Simulation by Computational Fluid Dynamic Analysis ,Energies (Basel) , Vol. 9 (11),pp 930 , , https://doi.org/https://doi.org/10.3390/en9110930
, Future energy-optimised buildings — Addressing the impact of climate change on buildings ,Energy Build , Vol. 231 ,pp 110610 , , https://doi.org/https://doi.org/10.1016/j.enbuild.2020.110610
, Technological options and strategies towards zero energy buildings contributing to climate change mitigation: A systematic review ,Energy Build , Vol. 219 ,pp 110009 , , https://doi.org/https://doi.org/10.1016/j.enbuild.2020.110009
, Environmental and economic implications of energy efficiency in new residential buildings: A multi-criteria selection approach ,Energy Strategy Reviews , Vol. 26 ,pp 100412 , , https://doi.org/https://doi.org/10.1016/j.esr.2019.100412
, A systematic review of recent air source heat pump (ASHP) systems assisted by solar thermal, photovoltaic and photovoltaic/thermal sources ,Renew Energy , Vol. 146 ,pp 2472–2487 , , https://doi.org/https://doi.org/10.1016/j.renene.2019.08.096
, The impact of climate change on air source heat pumps ,Energy Conversion and Management , Vol. 276 ,pp 116554 , 2023, https://doi.org/https://doi.org/10.1016/j.enconman.2022.116554
, Techno-economic analysis of air source heat pump applied for space heating in northern China ,Appl Energy , Vol. 207 ,pp 533–542 , , https://doi.org/https://doi.org/10.1016/j.apenergy.2017.06.083
, Seasonal thermal energy storage with heat pumps and low temperatures in building projects—A comparative review ,Renewable and Sustainable Energy Reviews , Vol. 43 ,pp 1199–1213 , , https://doi.org/https://doi.org/10.1016/j.rser.2014.12.002
, Performance Analysis of Air Cooled Heat Pump Coupled with Horizontal Air Ground Heat Exchanger in the Mediterranean Climate ,Energies (Basel) , Vol. 11 (10),pp 2704 , , https://doi.org/https://doi.org/10.3390/en11102704
, Numerical and experimental analysis of the energy performance of an air-source heat pump (ASHP) coupled with a horizontal earth-to-air heat exchanger (EAHX) in different climates ,Geothermics , Vol. 87 ,pp 101845 , , https://doi.org/https://doi.org/10.1016/j.geothermics.2020.101845
, Impact of global warming on performance of ground source heat pumps in US climate zones ,Energy Convers Manag , Vol. 101 ,pp 632–643 , , https://doi.org/https://doi.org/10.1016/j.enconman.2015.06.027
, Critical Analysis of Software Tools Aimed at Generating Future Weather Files with a view to their use in Building Performance Simulation ,Energy Procedia , Vol. 132 ,pp 640–645 , , https://doi.org/https://doi.org/10.1016/j.egypro.2017.09.701
, Constructing design weather data for future climates ,Building Services Engineering Research and Technology , Vol. 26 (1),pp 49–61 , , https://doi.org/https://doi.org/10.1191/0143624405bt112oa
, Transforming existing weather data for worldwide locations to enable energy and building performance simulation under future climates ,Renew Energy , Vol. 55 ,pp 514–524 , , https://doi.org/https://doi.org/10.1016/j.renene.2012.12.049
, Climate change future proofing of buildings—Generation and assessment of building simulation weather files ,Energy Build , Vol. 40 (12),pp 2148–2168 , , https://doi.org/https://doi.org/10.1016/j.enbuild.2008.06.005
,